Raman and near-infrared (NIR) spectroscopy are complementary methods, both probing vibrational transitions in molecules. In general, the strong bands in the (N)IR spectrum of a compound correspond to weak bands in the Raman and vice versa. This blog will look at some of the differences between Raman (light-scattering) and NIR (light absorption) methods.
Here just for the meat? Skip down to the “Cutting to the Chase” for some practical similarities and differences further below.
Quick Refresh: What is Vibrational Spectroscopy
Atoms aren’t wallflowers, they dance to the good vibrations!
When energy of the frequency range between 10,000 cm-1 and 100 cm-1 is absorbed by a molecule, it is converted into energy of molecular vibration. A stretching vibration occurs when the interatomic distance between two atoms increases or decreases along the bond axis, while bending vibrations are characterized by a change in the angle between two bonds with a common atom or the movement of a group of atoms with respect to the remainder of the molecule. The frequency or wavelength of absorption at which a vibration occurs depends on the relative mass of the atoms, the force constants of the bonds (i.e., bond strength), and the geometry of the atoms. Therefore, certain groups of atoms, or functional groups, give rise to characteristic peaks at or near the same frequency regardless of molecular structure; this is the premise for compound identification using vibrational spectroscopy. You can read more about this in a previous blog.
Take a look at some examples of Raman and NIR spectra (below) before we dig into some enlightening details about Raman spectroscopy.
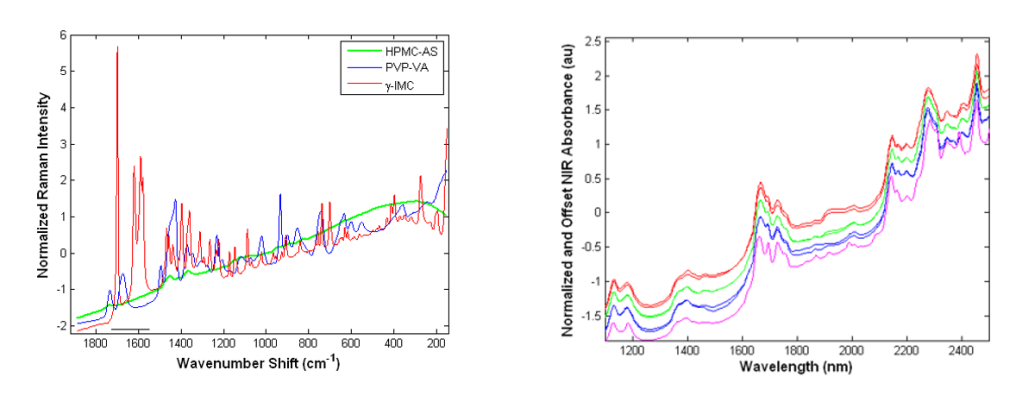
Raman: Digging into the Principles
Raman spectra are acquired by irradiating a sample with a monochromatic source (think: lasers) at a frequency (or energy) which is higher than the resonant vibrational frequencies of a molecule. Raman activity requires that the incident photons cause a transient distortion of the electron distribution of molecular bonds—a change in polarizability—which results in photon absorption. The absorption process promotes electrons to a virtual, non-quantized energy level, from which photons are reemitted (i.e., scattered) and detected. In general, atoms in which electrons are far from the sphere of influence of the positively charged nucleus are more polarizable (i.e. homonuclear bonds which experience symmetric vibrations).
Raman radiation may be elastically or inelastically scattered. When excitation occurs from the ground vibration energy level (v = 0), the inelastically scattered radiation is of a lower frequency than the excitation frequency, h(vex – vv), where vv is the vibrational frequency of the bond. This is called Stokes scattering. When molecules in the first excited vibrational state (v = 1) scatter radiation inelastically, the Raman signal produced is of energy h(vex + vv); the scattering occurs at a higher frequency than the radiation source and is called anti-Stokes scattering. Elastic scattering occurs when a photon is emitted with the same energy as the excitation source (hvex), called Rayleigh scattering. Only inelastically scattered photons provide information regarding molecular vibrations, although Stokes emission is generally favored for data analysis due to the higher signal intensities that are achieved relative to anti-Stokes scattering, a consequence of a greater population of electrons in the ground state at any given time relative to the excited state.
Raman spectra are recorded according to the wavenumber shift from the excitation frequency which is equivalent to the vibrational frequency. Therefore, the change in energy produced by the vibration would be equivalent to that observed in absorption if the bond was also IR-active, making the Raman frequency shift and the IR absorption frequency identical. Raman shift is independent of the excitation wavelength. The intensity of a Raman band depends on the polarizability of the molecule, the source intensity, and the concentration of the active group. Raman intensity is directly proportional to the concentration of the analyte.
Cutting to the Chase:
What are notable practical similarities between NIR and Raman?
- Both are used in chemistry to provide a structural fingerprint by which molecules can be identified
- Multivariate spectroscopic data analysis of Raman or NIR signal can be used to accomplish quantitative (compositional) analysis of organic samples
- They are both non-destructive, enabling direct analysis of intact samples (i.e. no dissolution, trituration or dilution of samples)
- Fast measurements (on the order of seconds to a few minutes) make these methods practical for higher frequency sampling or process monitoring
- Both spectrometer types may be interfaced with probes to improve sample accessibility, which is useful for process analytical technology applications
What are the practical differences between NIR and Raman
- If a molecule has a center of symmetry, Raman active vibrations would not be visible in the infrared. Asymmetric stretches, which induce a dipole, are infrared active. This difference is why Raman and Infrared Spectra are considered complementary
- Vibrations involving polar bonds (e.g. C-O, N-O, O-H) are weak Raman scatterers but strong NIR absorbers, while bonds such as C-C, C-H and C=C are observed well using Raman and not using NIR
- NIR spectra is more broad and overlapping than Raman, which is characterized by sharper peaks (signal). While multivariate analysis (e.g. PLS, PCA) often overcomes this challenge, Raman may offer better selectivity in some cases and is more appropriate for analysis of unknowns
- Whereas water is a strong absorber in NIR, it is inactive in Raman. Therefore, Raman analysis may provide an advantage when water is a major interference and not an analyte of interest (e.g. monitoring crystallization in aqueous solutions)
- When water is an analyte of interest (e.g. when monitoring a drying process), NIR is appropriate
- NIR is preferred for non-crystalline, hydrophilic molecules (like pharmaceutical excipients) which are often poor Raman-scatterers relative to the generally small aromatic, heterocyclic drug molecules
- Some Raman lasers may cause sample heating which may result in chemical degradation. Laser excitation may also cause sample fluorescence or photobleaching, which imparts baseline effects. This may be observed most in noncrystalline (amorphous) samples
You can learn more about BUCHI NIRSolutions, including: the flexible R&D laboratory NIRFlex N-500, the rugged at-line ProxiMate, and the in-line or on-line NIR-Online using the links.
Want to dig in deeper into the NIR vs Raman comparison? Check out an article published in Spectroscopy Magazine on the subject.
Leave a comment